Chapter 4: Matter and Energy in the Universe
Chapter 1
How Science Works
- The Scientific Method
- Evidence
- Measurements
- Units and the Metric System
- Measurement Errors
- Estimation
- Dimensions
- Mass, Length, and Time
- Observations and Uncertainty
- Precision and Significant Figures
- Errors and Statistics
- Scientific Notation
- Ways of Representing Data
- Logic
- Mathematics
- Geometry
- Algebra
- Logarithms
- Testing a Hypothesis
- Case Study of Life on Mars
- Theories
- Systems of Knowledge
- The Culture of Science
- Computer Simulations
- Modern Scientific Research
- The Scope of Astronomy
- Astronomy as a Science
- A Scale Model of Space
- A Scale Model of Time
- Questions
Chapter 2
Early Astronomy
- The Night Sky
- Motions in the Sky
- Navigation
- Constellations and Seasons
- Cause of the Seasons
- The Magnitude System
- Angular Size and Linear Size
- Phases of the Moon
- Eclipses
- Auroras
- Dividing Time
- Solar and Lunar Calendars
- History of Astronomy
- Stonehenge
- Ancient Observatories
- Counting and Measurement
- Astrology
- Greek Astronomy
- Aristotle and Geocentric Cosmology
- Aristarchus and Heliocentric Cosmology
- The Dark Ages
- Arab Astronomy
- Indian Astronomy
- Chinese Astronomy
- Mayan Astronomy
- Questions
Chapter 3
The Copernican Revolution
- Ptolemy and the Geocentric Model
- The Renaissance
- Copernicus and the Heliocentric Model
- Tycho Brahe
- Johannes Kepler
- Elliptical Orbits
- Kepler's Laws
- Galileo Galilei
- The Trial of Galileo
- Isaac Newton
- Newton's Law of Gravity
- The Plurality of Worlds
- The Birth of Modern Science
- Layout of the Solar System
- Scale of the Solar System
- The Idea of Space Exploration
- Orbits
- History of Space Exploration
- Moon Landings
- International Space Station
- Manned versus Robotic Missions
- Commercial Space Flight
- Future of Space Exploration
- Living in Space
- Moon, Mars, and Beyond
- Societies in Space
- Questions
Chapter 5
The Earth-Moon System
- Earth and Moon
- Early Estimates of Earth's Age
- How the Earth Cooled
- Ages Using Radioactivity
- Radioactive Half-Life
- Ages of the Earth and Moon
- Geological Activity
- Internal Structure of the Earth and Moon
- Basic Rock Types
- Layers of the Earth and Moon
- Origin of Water on Earth
- The Evolving Earth
- Plate Tectonics
- Volcanoes
- Geological Processes
- Impact Craters
- The Geological Timescale
- Mass Extinctions
- Evolution and the Cosmic Environment
- Earth's Atmosphere and Oceans
- Weather Circulation
- Environmental Change on Earth
- The Earth-Moon System
- Geological History of the Moon
- Tidal Forces
- Effects of Tidal Forces
- Historical Studies of the Moon
- Lunar Surface
- Ice on the Moon
- Origin of the Moon
- Humans on the Moon
- Questions
Chapter 6
The Terrestrial Planets
- Studying Other Planets
- The Planets
- The Terrestrial Planets
- Mercury
- Mercury's Orbit
- Mercury's Surface
- Venus
- Volcanism on Venus
- Venus and the Greenhouse Effect
- Tectonics on Venus
- Exploring Venus
- Mars in Myth and Legend
- Early Studies of Mars
- Mars Close-Up
- Modern Views of Mars
- Missions to Mars
- Geology of Mars
- Water on Mars
- Polar Caps of Mars
- Climate Change on Mars
- Terraforming Mars
- Life on Mars
- The Moons of Mars
- Martian Meteorites
- Comparative Planetology
- Incidence of Craters
- Counting Craters
- Counting Statistics
- Internal Heat and Geological Activity
- Magnetic Fields of the Terrestrial Planets
- Mountains and Rifts
- Radar Studies of Planetary Surfaces
- Laser Ranging and Altimetry
- Gravity and Atmospheres
- Normal Atmospheric Composition
- The Significance of Oxygen
- Questions
Chapter 7
The Giant Planets and Their Moons
- The Gas Giant Planets
- Atmospheres of the Gas Giant Planets
- Clouds and Weather on Gas Giant Planets
- Internal Structure of the Gas Giant Planets
- Thermal Radiation from Gas Giant Planets
- Life on Gas Giant Planets?
- Why Giant Planets are Giant
- Gas Laws
- Ring Systems of the Giant Planets
- Structure Within Ring Systems
- The Origin of Ring Particles
- The Roche Limit
- Resonance and Harmonics
- Tidal Forces in the Solar System
- Moons of Gas Giant Planets
- Geology of Large Moons
- The Voyager Missions
- Jupiter
- Jupiter's Galilean Moons
- Jupiter's Ganymede
- Jupiter's Europa
- Jupiter's Callisto
- Jupiter's Io
- Volcanoes on Io
- Saturn
- Cassini Mission to Saturn
- Saturn's Titan
- Saturn's Enceladus
- Discovery of Uranus and Neptune
- Uranus
- Uranus' Miranda
- Neptune
- Neptune's Triton
- Pluto
- The Discovery of Pluto
- Pluto as a Dwarf Planet
- Dwarf Planets
- Questions
Chapter 8
Interplanetary Bodies
- Interplanetary Bodies
- Comets
- Early Observations of Comets
- Structure of the Comet Nucleus
- Comet Chemistry
- Oort Cloud and Kuiper Belt
- Kuiper Belt
- Comet Orbits
- Life Story of Comets
- The Largest Kuiper Belt Objects
- Meteors and Meteor Showers
- Gravitational Perturbations
- Asteroids
- Surveys for Earth Crossing Asteroids
- Asteroid Shapes
- Composition of Asteroids
- Introduction to Meteorites
- Origin of Meteorites
- Types of Meteorites
- The Tunguska Event
- The Threat from Space
- Probability and Impacts
- Impact on Jupiter
- Interplanetary Opportunity
- Questions
Chapter 9
Planet Formation and Exoplanets
- Formation of the Solar System
- Early History of the Solar System
- Conservation of Angular Momentum
- Angular Momentum in a Collapsing Cloud
- Helmholtz Contraction
- Safronov and Planet Formation
- Collapse of the Solar Nebula
- Why the Solar System Collapsed
- From Planetesimals to Planets
- Accretion and Solar System Bodies
- Differentiation
- Planetary Magnetic Fields
- The Origin of Satellites
- Solar System Debris and Formation
- Gradual Evolution and a Few Catastrophies
- Chaos and Determinism
- Extrasolar Planets
- Discoveries of Exoplanets
- Doppler Detection of Exoplanets
- Transit Detection of Exoplanets
- The Kepler Mission
- Direct Detection of Exoplanets
- Properties of Exoplanets
- Implications of Exoplanet Surveys
- Future Detection of Exoplanets
- Questions
Chapter 10
Detecting Radiation from Space
- Observing the Universe
- Radiation and the Universe
- The Nature of Light
- The Electromagnetic Spectrum
- Properties of Waves
- Waves and Particles
- How Radiation Travels
- Properties of Electromagnetic Radiation
- The Doppler Effect
- Invisible Radiation
- Thermal Spectra
- The Quantum Theory
- The Uncertainty Principle
- Spectral Lines
- Emission Lines and Bands
- Absorption and Emission Spectra
- Kirchoff's Laws
- Astronomical Detection of Radiation
- The Telescope
- Optical Telescopes
- Optical Detectors
- Adaptive Optics
- Image Processing
- Digital Information
- Radio Telescopes
- Telescopes in Space
- Hubble Space Telescope
- Interferometry
- Collecting Area and Resolution
- Frontier Observatories
- Questions
Chapter 11
Our Sun: The Nearest Star
- The Sun
- The Nearest Star
- Properties of the Sun
- Kelvin and the Sun's Age
- The Sun's Composition
- Energy From Atomic Nuclei
- Mass-Energy Conversion
- Examples of Mass-Energy Conversion
- Energy From Nuclear Fission
- Energy From Nuclear Fusion
- Nuclear Reactions in the Sun
- The Sun's Interior
- Energy Flow in the Sun
- Collisions and Opacity
- Solar Neutrinos
- Solar Oscillations
- The Sun's Atmosphere
- Solar Chromosphere and Corona
- Sunspots
- The Solar Cycle
- The Solar Wind
- Effects of the Sun on the Earth
- Cosmic Energy Sources
- Questions
Chapter 12
Properties of Stars
- Stars
- Star Names
- Star Properties
- The Distance to Stars
- Apparent Brightness
- Absolute Brightness
- Measuring Star Distances
- Stellar Parallax
- Spectra of Stars
- Spectral Classification
- Temperature and Spectral Class
- Stellar Composition
- Stellar Motion
- Stellar Luminosity
- The Size of Stars
- Stefan-Boltzmann Law
- Stellar Mass
- Hydrostatic Equilibrium
- Stellar Classification
- The Hertzsprung-Russell Diagram
- Volume and Brightness Selected Samples
- Stars of Different Sizes
- Understanding the Main Sequence
- Stellar Structure
- Stellar Evolution
- Questions
Chapter 13
Star Birth and Death
- Star Birth and Death
- Understanding Star Birth and Death
- Cosmic Abundance of Elements
- Star Formation
- Molecular Clouds
- Young Stars
- T Tauri Stars
- Mass Limits for Stars
- Brown Dwarfs
- Young Star Clusters
- Cauldron of the Elements
- Main Sequence Stars
- Nuclear Reactions in Main Sequence Stars
- Main Sequence Lifetimes
- Evolved Stars
- Cycles of Star Life and Death
- The Creation of Heavy Elements
- Red Giants
- Horizontal Branch and Asymptotic Giant Branch Stars
- Variable Stars
- Magnetic Stars
- Stellar Mass Loss
- White Dwarfs
- Supernovae
- Seeing the Death of a Star
- Supernova 1987A
- Neutron Stars and Pulsars
- Special Theory of Relativity
- General Theory of Relativity
- Black Holes
- Properties of Black Holes
- Questions
Chapter 14
The Milky Way
- The Distribution of Stars in Space
- Stellar Companions
- Binary Star Systems
- Binary and Multiple Stars
- Mass Transfer in Binaries
- Binaries and Stellar Mass
- Nova and Supernova
- Exotic Binary Systems
- Gamma Ray Bursts
- How Multiple Stars Form
- Environments of Stars
- The Interstellar Medium
- Effects of Interstellar Material on Starlight
- Structure of the Interstellar Medium
- Dust Extinction and Reddening
- Groups of Stars
- Open Star Clusters
- Globular Star Clusters
- Distances to Groups of Stars
- Ages of Groups of Stars
- Layout of the Milky Way
- William Herschel
- Isotropy and Anisotropy
- Mapping the Milky Way
- Questions
Chapter 15
Galaxies
- The Milky Way Galaxy
- Mapping the Galaxy Disk
- Spiral Structure in Galaxies
- Mass of the Milky Way
- Dark Matter in the Milky Way
- Galaxy Mass
- The Galactic Center
- Black Hole in the Galactic Center
- Stellar Populations
- Formation of the Milky Way
- Galaxies
- The Shapley-Curtis Debate
- Edwin Hubble
- Distances to Galaxies
- Classifying Galaxies
- Spiral Galaxies
- Elliptical Galaxies
- Lenticular Galaxies
- Dwarf and Irregular Galaxies
- Overview of Galaxy Structures
- The Local Group
- Light Travel Time
- Galaxy Size and Luminosity
- Mass to Light Ratios
- Dark Matter in Galaxies
- Gravity of Many Bodies
- Galaxy Evolution
- Galaxy Interactions
- Galaxy Formation
- Questions
Chapter 16
The Expanding Universe
- Galaxy Redshifts
- The Expanding Universe
- Cosmological Redshifts
- The Hubble Relation
- Relating Redshift and Distance
- Galaxy Distance Indicators
- Size and Age of the Universe
- The Hubble Constant
- Large Scale Structure
- Galaxy Clustering
- Clusters of Galaxies
- Overview of Large Scale Structure
- Dark Matter on the Largest Scales
- The Most Distant Galaxies
- Black Holes in Nearby Galaxies
- Active Galaxies
- Radio Galaxies
- The Discovery of Quasars
- Quasars
- Types of Gravitational Lensing
- Properties of Quasars
- The Quasar Power Source
- Quasars as Probes of the Universe
- Star Formation History of the Universe
- Expansion History of the Universe
- Questions
Chapter 17
Cosmology
- Cosmology
- Early Cosmologies
- Relativity and Cosmology
- The Big Bang Model
- The Cosmological Principle
- Universal Expansion
- Cosmic Nucleosynthesis
- Cosmic Microwave Background Radiation
- Discovery of the Microwave Background Radiation
- Measuring Space Curvature
- Cosmic Evolution
- Evolution of Structure
- Mean Cosmic Density
- Critical Density
- Dark Matter and Dark Energy
- Age of the Universe
- Precision Cosmology
- The Future of the Contents of the Universe
- Fate of the Universe
- Alternatives to the Big Bang Model
- Space-Time
- Particles and Radiation
- The Very Early Universe
- Mass and Energy in the Early Universe
- Matter and Antimatter
- The Forces of Nature
- Fine-Tuning in Cosmology
- The Anthropic Principle in Cosmology
- String Theory and Cosmology
- The Multiverse
- The Limits of Knowledge
- Questions
Chapter 18
Life On Earth
- Nature of Life
- Chemistry of Life
- Molecules of Life
- The Origin of Life on Earth
- Origin of Complex Molecules
- Miller-Urey Experiment
- Pre-RNA World
- RNA World
- From Molecules to Cells
- Metabolism
- Anaerobes
- Extremophiles
- Thermophiles
- Psychrophiles
- Xerophiles
- Halophiles
- Barophiles
- Acidophiles
- Alkaliphiles
- Radiation Resistant Biology
- Importance of Water for Life
- Hydrothermal Systems
- Silicon Versus Carbon
- DNA and Heredity
- Life as Digital Information
- Synthetic Biology
- Life in a Computer
- Natural Selection
- Tree Of Life
- Evolution and Intelligence
- Culture and Technology
- The Gaia Hypothesis
- Life and the Cosmic Environment
Chapter 19
Life in the Universe
- Life in the Universe
- Astrobiology
- Life Beyond Earth
- Sites for Life
- Complex Molecules in Space
- Life in the Solar System
- Lowell and Canals on Mars
- Implications of Life on Mars
- Extreme Environments in the Solar System
- Rare Earth Hypothesis
- Are We Alone?
- Unidentified Flying Objects or UFOs
- The Search for Extraterrestrial Intelligence
- The Drake Equation
- The History of SETI
- Recent SETI Projects
- Recognizing a Message
- The Best Way to Communicate
- The Fermi Question
- The Anthropic Principle
- Where Are They?
Laws of Thermodynamics
Energy behaves in regular and predictable ways in the universe. Thermodynamics is the study of the way that heat flows. We can generalize that discussion to include heat and all other forms of energy. The rules that scientists have discovered, applying to every region of the universe looked at so far, are called the laws of thermodynamics.
Although not one of the traditional three laws, physicists define a zeroth law of thermodynamics. It defines thermal equilibrium as the situation when there is no flow of energy between two physical systems. Also, if two systems are each in thermal equilibrium with a third system, then they are in thermal equilibrium with each other. The zeroth law forms the basis for the definition of temperature.
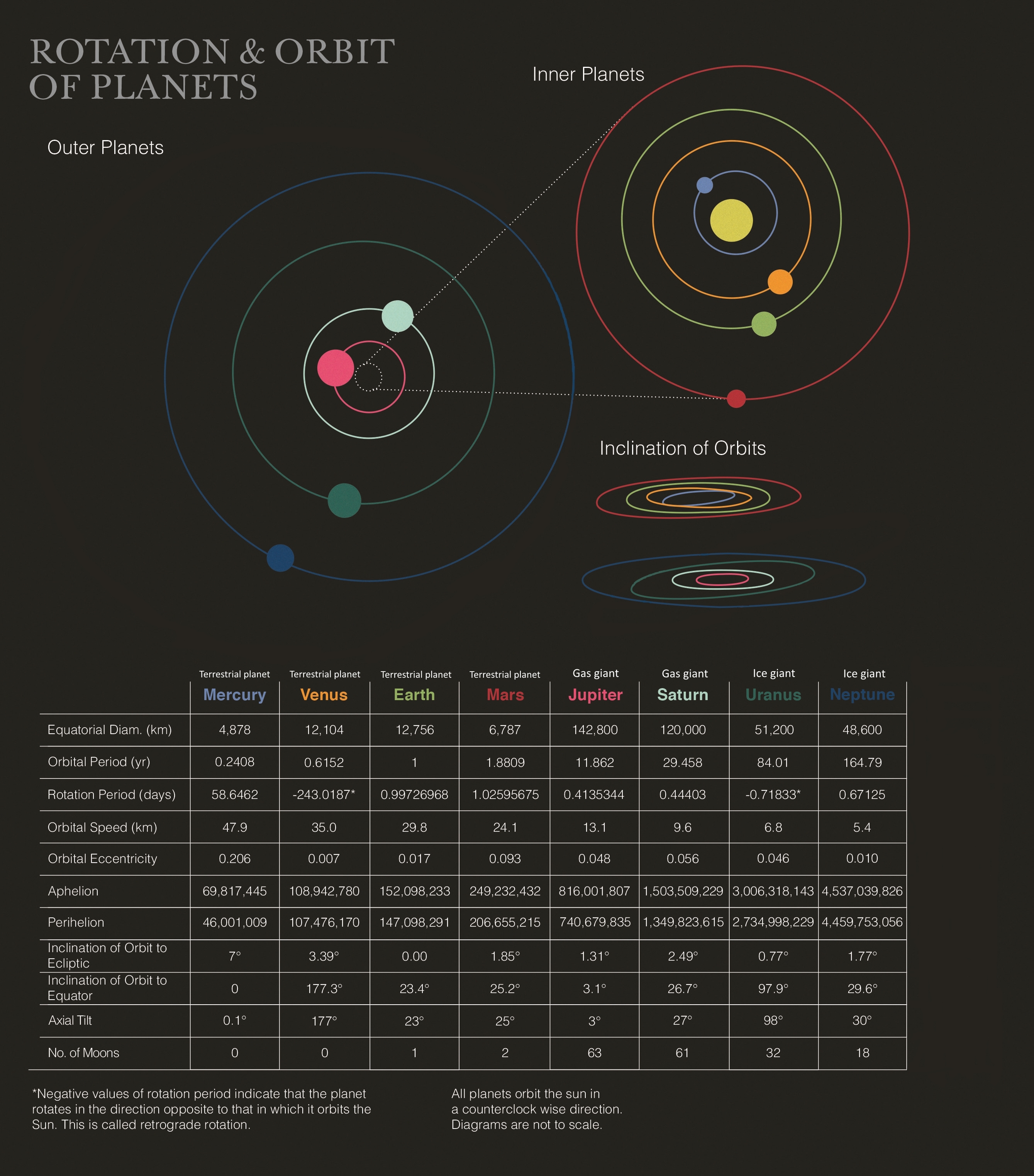
The first law of thermodynamics states that the total amount of energy in a closed system is always conserved. This is a statement of the law of conservation of energy. For example, an elliptical orbit has a constant interplay between kinetic energy and gravitational potential energy. When it is close to its star, a planet moves faster in its orbit — the kinetic energy is high and the gravitational energy is low. When it is far from its star, a planet moves slower in its orbit — the kinetic energy is low and the gravitational energy is high. The total energy of the planet remains constant. When energy appears not to be conserved, the answer is usually that some energy has leaked away in the form of heat. When a swing slows down or a rolling ball comes to a stop, kinetic energy has steadily been converted into heat.
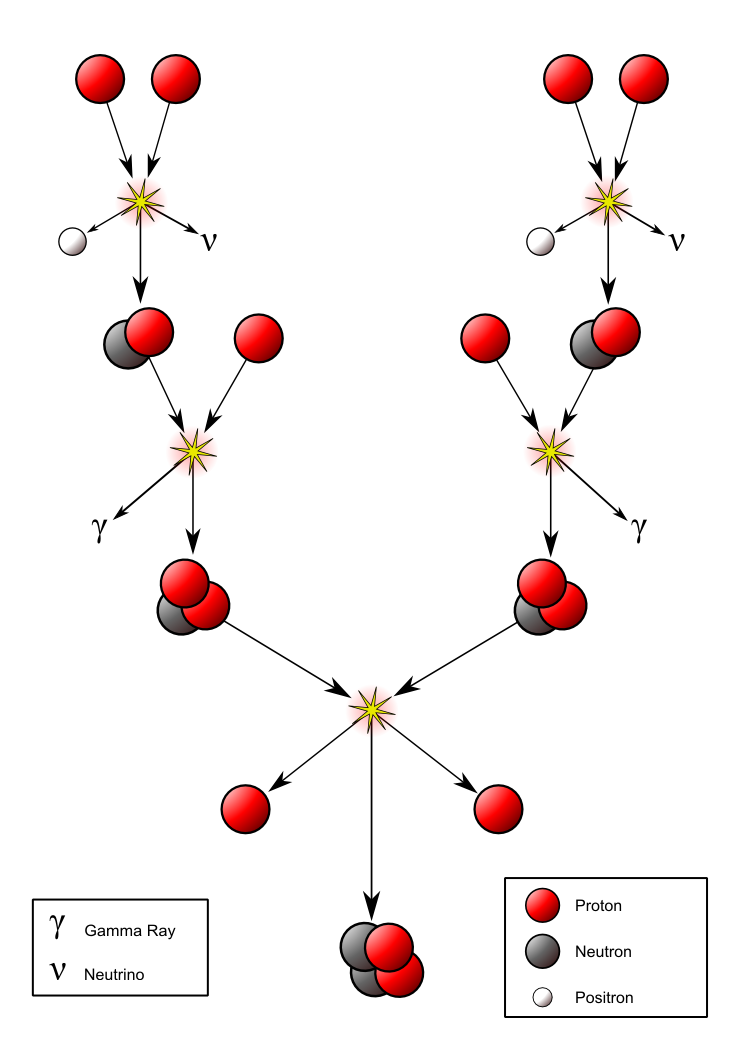
When we look at the Sun blazing in the sky and consider that it will keep doing so for billions of years, it seems as if we get this energy for free. In what way do stars conserve energy? Stars create heavier elements in a fusion chain that moves from hydrogen to helium and, if the star is massive enough, to carbon and on to iron. Atomic nuclei are bound together with an attractive force that acts like "glue." It takes energy to undo this glue. Since Einstein showed that the energy that binds an atomic nucleus must have an equivalent energy (E = mc2), it follows that things stuck together have less mass than the same things pulled apart. Therefore, whenever a nucleus becomes more tightly bound, the energy that has been frozen in the form of mass gets released as radiant energy. Going from hydrogen to iron, atomic nuclei are held together more and more tightly. So each step up the fusion chain releases mass-energy. Think of mass as a form of potential energy that stars convert into light.
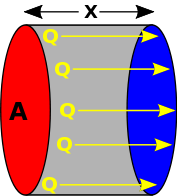
The second law of thermodynamics is also familiar. The principle of thermal equilibrium describes how heat always flows from a hot object to a cold object. Equilibrium is established when both objects have the same temperature. The second law makes the strong proposition: heat cannot flow spontaneously from a cold to a hot object. A cup of coffee cools down as it loses heat to the cooler atmosphere. An ice cube melts as heat flows into it from the warmer atmosphere. Notice that the first law, conservation of energy, does not require this behavior. Energy would be conserved if coffee actually got hotter at the expense of cooling the air, or if an ice cube got colder at the expense of warming the air. But we never see this happen. In nature, heat always flows in one direction from hot to cold.
When the second law of thermodynamics appears to be violated, it is because we are not considering the whole system. Heat always flows so as to warm things up, but that doesn't mean we can't make ice cubes. A refrigerator can extract enough heat from water to make ice cubes, but it comes at the expense of releasing a larger amount of heat into the air. Feel along the top and bottom of your refrigerator if you don't believe this. It is easy to see that stars follow the second law. Energy is created in the core of a star by nuclear fusion and then it flows out from hotter to cooler regions.
It is possible to express the second law of thermodynamics uses a microscopic description of heat energy. Heat is a measure of the kinetic energy (or amount of motion) of atoms and molecules. Fast-moving particles tend to share their motion with slower-moving particles, and the result is that they all approach the same motion or temperature. This is the idea of thermal equilibrium. Notice two important features of this description. First, it is possible for a slow-moving particle to give up speed to a fast-moving particle, but it is unlikely to happen. When considering a large number of particles, the energy will always flow from the quick to the slow. The second law of thermodynamics describes nature with probability rather than with certainty. A few water molecules might happen to lose enough energy to stick together as an ice crystal, but the chances of an ice cube spontaneously forming in your glass of water are incredibly small!
The second consequence of the microscopic description of heat flow is even more interesting. Heat is a measure of the random motions of particles, so it is really a measure of disorder. The second law of thermodynamics also states that the disorder of a physical system will always increase when it undergoes changes. Scientists use the term entropy as a measure of disorder. Entropy is a profound scientific concept, useful in many areas of astronomy.
The increase of entropy seems to be a fact of life as well as a law of nature. The papers on your desk tend to get disordered, odd socks turn up in your sock drawer, and the kitchen always seems to get messy. Why is it so much easier to break an egg than to put it back together? Why can you easily stir a sugar cube into your coffee, while no amount of stirring will make the sugar cube come back together? Let's use the example of a deck of cards. Start with a deck where all the cards are ranked by number and suit. The deck is perfectly ordered and it has low entropy. Anything you do to the deck will make it less ordered. After a few shuffles, there will be little groups of cards in sequence, but overall the deck will have more disorder and more entropy. Shuffle many times and the deck will begin to look truly random; the disorder and entropy are now very high. Experience tells you that no matter how often you shuffle the deck, you will never return the cards to their initial, ordered state. Entropy always increases.
There is a clear connection between disorder or entropy and the probability of a system being in a certain state. Situations of disorder are more probable than situations of order. When an ice cube (or a sugar cube) melts, the order in the crystal lattice turns into the disorder of randomly moving water molecules. Heat is a random or high entropy form of energy. Change is all around us, and energy is always changing from one form to another. The first law says that energy is conserved. The second law says that energy tends to transform into disordered energy or heat.
Ordered energy changes systematically into disordered energy, as we can see with many everyday examples. The motion of a swing or a pendulum decays, and the lost kinetic energy turns into heat energy in the slight heating of air molecules. Our world runs on fossil fuel, which taps the energy stored in the structure of the chemical bond. No matter how efficiently we use that energy, much of it is turned into heat. The electricity in your house heats the wires that it travels through, and later that heat leaks into the atmosphere. A magnet loses its strength with time and use. In this case, the aligned iron grains gradually become less aligned due to heat and due to the magnet striking other objects. (This also creates heat.) Magnetic energy is converted into heat energy. What does this have to do with stars? A star converts mass into energy. This is a very direct translation of order, in the form of a localized particle, into disorder, in the form of radiation or heat energy that extends through space.
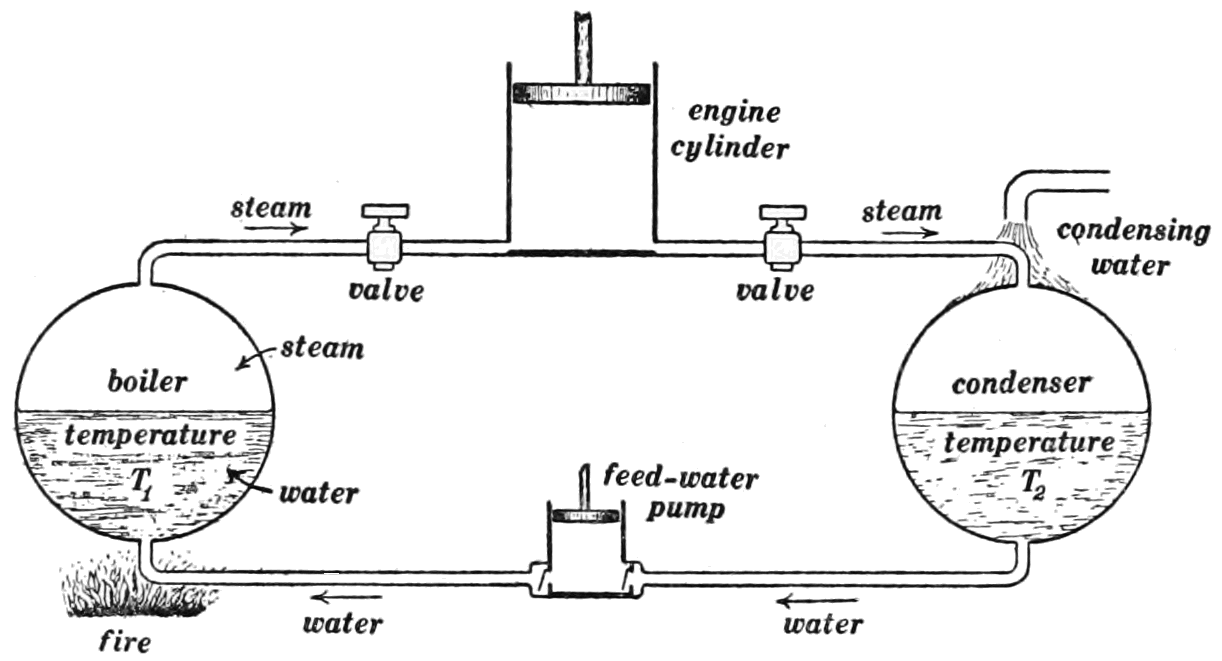
In any case, where the second law appears to be violated, we are not looking at the whole picture. A refrigerator can make ice cubes, creating the order of a crystal lattice, but only at the expense of releasing a far larger amount of disordered (heat) energy. A star can build heavy elements, creating the structure of a massive nucleus, but only at the expense of disordered (radiation) energy sent into space. How does life comply with the second law of thermodynamics? Surely the creation of the structure of a brain, or a cell, or DNA itself, violates the trend toward disorder? It is true that living organisms have lower entropy than their surroundings. However, in the microscopic view, many scientists think that aging is caused by the gradual accumulation of damage to the DNA molecule. This increasing disorder in the genetic code compromises the operation of cells and limits the ability of the organism to repair itself. And in the larger view, the Earth intercepts only a tiny fraction of the Sun's heat energy. Life eventually dies and returns that energy to the atmosphere and then into deep space as a waste product. The second law is obeyed.
Physicists have identified another principle that is often called the third law of thermodynamics. It states that no physical system can be cooled to a temperature of absolute zero. Think of it — at absolute zero there would be no atomic motion so there would be no heat and no friction. Machines could be perfectly efficient. Perpetual motion would be possible. But it is impossible to remove all the heat from a system. Take the coldest gas you can imagine. If you expand its container the gas will cool. But you would have to expand it by an infinite amount to completely remove its heat content. Absolute zero cannot be reached.
The three laws of thermodynamics can be summarized as follows:
• Energy can change forms, but the total amount of energy in a system, including heat energy, is always conserved.
• The entropy, or disorder of a system, always increases, so as energy changes forms, the amount of heat energy tends to increase.
• It is impossible to remove all the heat from a physical system.
Here is a colloquial aid to memorizing these important laws. Think of the exchange of heat and other forms of energy as a game. In physics, it turns out you can't get something for nothing. The first law says you can't win. The second law says you can't break even. Worst of all, the third law says you can't get out of the game!